Exodus propulsion technologies represent humanity’s ambitious quest for interstellar travel. This exploration delves into the diverse and fascinating array of propulsion methods being considered, from established technologies like ion propulsion to the more theoretical, yet captivating, concepts of antimatter propulsion and even warp drives. We will examine the scientific principles, technological challenges, and potential solutions associated with each, offering a comprehensive overview of the current state of the field and the future prospects of interstellar voyages.
The journey to interstellar travel demands innovation far beyond our current capabilities. This necessitates a multi-faceted approach, encompassing not only advancements in propulsion systems but also breakthroughs in materials science, energy storage, and life support technologies. We will explore the intricate interplay of these factors, examining how they contribute to the overall feasibility and timeline for achieving interstellar flight.
Introduction to Exodus Propulsion
The concept of “Exodus Propulsion” refers to propulsion systems capable of enabling interstellar travel, facilitating the potential migration of humanity to other star systems. This necessitates speeds far exceeding those currently achievable, requiring breakthroughs in fundamental physics and engineering. The historical pursuit of such capabilities is a fascinating journey marked by both incremental progress and significant hurdles.
The development of advanced propulsion systems has been intrinsically linked to humanity’s expanding understanding of the universe and our place within it. From early dreams of reaching the stars to the sophisticated calculations underpinning modern space exploration, the desire to overcome the vast distances separating celestial bodies has driven innovation. This drive has been fueled by a combination of scientific curiosity, the search for habitable planets, and even the need for a “Plan B” in the face of potential existential threats to Earth.
A Timeline of Significant Advancements
The journey towards Exodus Propulsion is a long and winding one. Early efforts focused on incremental improvements to chemical rockets, which, while effective for near-Earth missions, fall drastically short of the speeds required for interstellar travel. Significant milestones include the development of liquid-fueled rockets in the early 20th century, marking a crucial step towards more powerful and efficient propulsion. The subsequent development of solid-propellant rockets provided a more readily deployable, albeit less efficient, alternative. The Space Race of the 1960s spurred rapid advancements, culminating in the Apollo missions to the Moon, showcasing the potential of powerful, albeit still chemically-based, rockets. However, even with these advancements, interstellar travel remained firmly in the realm of science fiction.
More recently, research has focused on alternative propulsion concepts such as ion propulsion, nuclear thermal propulsion, and even more speculative ideas like antimatter propulsion and warp drives. Ion propulsion systems, already used in some spacecraft, offer higher specific impulse (a measure of fuel efficiency) than chemical rockets. Nuclear thermal propulsion promises significantly greater thrust, but presents challenges related to safety and radiation shielding. Antimatter propulsion, while theoretically capable of incredibly high speeds, faces immense technological hurdles related to antimatter production, storage, and control. The concept of warp drives, inspired by science fiction, remains purely theoretical, requiring breakthroughs in our understanding of spacetime.
Historical Context and Motivations
The quest for advanced propulsion has been interwoven with broader societal and scientific trends. Early science fiction significantly shaped public imagination, fueling interest in space exploration and interstellar travel. The post-World War II era saw a surge in government investment in space research, driven by both scientific ambition and geopolitical competition. The discovery of exoplanets, starting in the 1990s, provided further impetus, offering the tantalizing possibility of finding habitable worlds beyond our solar system. The growing awareness of potential threats to Earth, such as asteroid impacts or climate change, has also contributed to the urgency of developing interstellar travel capabilities as a potential safeguard for humanity’s long-term survival.
Challenges and Limitations of Past Attempts
The development of Exodus-style propulsion faces numerous challenges. The sheer energy requirements for interstellar travel are immense. Even with highly efficient propulsion systems, the journey to the nearest star system would take decades, if not centuries, using currently conceivable technologies. Furthermore, the engineering complexities associated with designing and building spacecraft capable of withstanding the rigors of interstellar travel are significant. Issues such as radiation shielding, life support systems for long-duration missions, and the potential for equipment failure during such long journeys pose considerable hurdles. Finally, the financial costs associated with such ambitious projects are astronomical, requiring significant international collaboration and sustained investment. The development of advanced materials, more efficient energy sources, and a deeper understanding of fundamental physics are all crucial for overcoming these limitations.
Nuclear Fusion Propulsion for Exodus
Nuclear fusion, the process that powers the sun, holds immense potential for interstellar travel. Its high energy density offers the possibility of achieving speeds and travel times far exceeding those achievable with current chemical or even fission-based propulsion systems. This section will explore a conceptual fusion-powered spacecraft design, compare different reactor types, and address the significant challenges associated with harnessing this powerful energy source for propulsion.
Conceptual Model of a Fusion-Powered Interstellar Spacecraft
A viable fusion-powered spacecraft for interstellar travel would require a multi-faceted design incorporating advanced technologies. Imagine a vessel several kilometers in length, with a modular design allowing for expansion and adaptation during the long voyage. The core would house the fusion reactor, shielded heavily to protect the crew from radiation. This reactor would be coupled to a magnetic nozzle system to direct the plasma exhaust, generating thrust. Power generation would also be integrated to support life support systems, communications, and onboard research facilities. Propulsion would be primarily based on the fusion reaction’s exhaust, though supplementary systems, like ion thrusters, might be used for course corrections and maneuvering. Efficient energy storage would be crucial, potentially utilizing advanced superconducting technologies to minimize energy loss during long-duration missions. The vessel would also need robust radiation shielding and advanced life support systems to ensure crew survival during the potentially decades-long journey.
Energy Source | Thrust Generation | Potential Limitations | Mitigation Strategies |
---|---|---|---|
Deuterium-Helium-3 fusion reaction (D-He3) offering high energy output and reduced neutron production compared to D-T reactions. | Magnetic nozzle directing the plasma exhaust, potentially supplemented by ion thrusters for fine control. | Reactor size and mass, maintaining plasma confinement, achieving sustained fusion reaction, and managing heat dissipation. | Advanced materials science for lighter, stronger reactor components; advanced plasma confinement techniques; robust cooling systems; and modular reactor design for redundancy and maintainability. |
Comparison of Fusion Reactor Designs for Interstellar Missions
Several fusion reactor designs are being researched, each with varying degrees of suitability for interstellar missions. The primary considerations include energy output, size, mass, and efficiency.
Aneutronic fusion reactions, such as D-He3, are particularly attractive due to their significantly reduced neutron production, minimizing radiation shielding requirements. However, achieving ignition with D-He3 requires significantly higher temperatures and pressures compared to the more established D-T reaction. Tokamak reactors, while well-researched, are typically large and complex. Inertial confinement fusion (ICF), using lasers or particle beams to ignite fuel pellets, could offer a more compact design, but faces challenges in achieving high repetition rates necessary for sustained thrust. Stellarators, offering potentially superior plasma confinement compared to tokamaks, are still in the relatively early stages of development. The optimal choice will likely depend on advancements in materials science, plasma physics, and engineering over the coming decades.
Solutions to the Challenges of Containing and Harnessing Fusion Energy for Propulsion
Containing and harnessing fusion energy for propulsion presents formidable challenges. The extreme temperatures and pressures involved demand materials with exceptional heat resistance and strength. Research into advanced materials, such as high-temperature superconductors and novel ceramics, is crucial. Improved plasma confinement techniques are also vital to maximize energy output and minimize energy loss. This involves sophisticated magnetic field control and potentially novel approaches like inertial electrostatic confinement. Efficient energy conversion from the fusion reaction to thrust requires advanced nozzle designs and plasma exhaust management. Developing effective and compact shielding against radiation produced during the fusion process is essential for crew safety. Finally, the development of reliable, autonomous systems for reactor operation and maintenance is critical for long-duration interstellar missions. These technological advancements are interconnected and require a concerted effort across multiple scientific and engineering disciplines.
Antimatter Propulsion Systems for Exodus
Antimatter propulsion represents a theoretical pinnacle in spacecraft propulsion, promising speeds and travel times far exceeding those achievable with current technologies. The concept hinges on the annihilation of matter and antimatter, releasing enormous amounts of energy in the process. While incredibly powerful, significant technological hurdles remain before antimatter propulsion can become a reality for missions like Project Exodus.
Antimatter, as its name suggests, is the opposite of ordinary matter. When matter and antimatter collide, they annihilate each other, converting their entire mass into energy according to Einstein’s famous equation, E=mc². This energy release is far more efficient than any chemical or nuclear reaction, offering the potential for exceptionally high specific impulse – a measure of a rocket engine’s efficiency.
Antimatter Propulsion System Design for Exodus
A hypothetical antimatter-powered spacecraft for Project Exodus would necessitate a multifaceted design addressing energy storage, propulsion, and crucially, safety. The spacecraft would require a sophisticated system for generating, storing, and controlling antimatter, likely involving magnetic confinement techniques to prevent premature annihilation. A plausible propulsion mechanism could involve a magnetic nozzle, directing the resulting charged particles from the annihilation reaction to generate thrust.
The energy storage system would need to manage vast amounts of antimatter, potentially utilizing a series of nested magnetic traps of increasing strength. Each trap would contain a progressively smaller amount of antimatter, with the innermost trap holding the quantity required for immediate propulsion. The entire system would be heavily shielded to protect against radiation. Safety protocols would be paramount, involving redundant systems for antimatter containment and emergency shutdown procedures to mitigate the risk of catastrophic annihilation. A sophisticated monitoring system would continuously track the antimatter’s state and any potential containment breaches.
Technological Hurdles in Antimatter Propulsion
Several significant technological hurdles must be overcome before antimatter propulsion becomes feasible. The primary challenge lies in the production and storage of antimatter. Currently, producing antimatter is extremely inefficient and energy-intensive, requiring vast amounts of energy to create minuscule quantities. Furthermore, storing antimatter safely for extended periods is a considerable engineering feat. The immense energy density of antimatter presents significant risks; any accidental contact with ordinary matter would lead to a powerful explosion. Finally, developing a reliable and efficient propulsion system capable of harnessing the energy released from matter-antimatter annihilation is a significant technological challenge. The design and construction of such a propulsion system would require materials capable of withstanding extreme temperatures and radiation levels. For instance, achieving the necessary containment fields and nozzle design would require advancements in materials science and electromagnetic engineering far beyond current capabilities. Research into high-temperature superconductors and advanced materials with extreme radiation resistance would be essential.
Ion Propulsion and its Role in Exodus
Ion propulsion offers a compelling solution for the immense distances involved in an exodus mission. Its high fuel efficiency, though coupled with low thrust, makes it ideally suited for long-duration, continuous acceleration scenarios, gradually building up velocity over extended periods. This contrasts sharply with chemical rockets, which deliver powerful but short bursts of thrust, consuming vast amounts of propellant in the process.
Ion propulsion relies on the principle of electrostatic acceleration. A propellant, typically a noble gas like xenon, is ionized—stripped of some of its electrons—creating positively charged ions. These ions are then accelerated by a strong electric field generated between charged grids or electrodes, creating a high-velocity ion beam that propels the spacecraft forward. The thrust produced is relatively low, but because ion thrusters can operate continuously for extended periods, they can achieve significantly higher final velocities compared to chemical rockets using the same propellant mass. This is particularly crucial for interstellar travel where even small increases in velocity can dramatically reduce travel times.
Ion Thruster Types and Performance Characteristics
Different types of ion thrusters exhibit varying levels of efficiency and thrust. Gridded ion thrusters, the most common type, use grids to accelerate ions. They are relatively mature technology, offering good efficiency and a reasonable level of thrust, though they can be susceptible to erosion over time. Hall-effect thrusters utilize a combination of electric and magnetic fields to ionize and accelerate the propellant, generally achieving higher thrust than gridded ion thrusters but with slightly lower efficiency. Electromagnetic thrusters, a more advanced concept, leverage magnetic fields to accelerate the propellant, potentially offering even higher thrust and efficiency but with increased technological complexity. A direct comparison of these thrusters is difficult as performance metrics vary significantly depending on design specifics and operating conditions; however, generally, gridded ion thrusters provide a balance between thrust and efficiency, while Hall-effect thrusters prioritize thrust, and electromagnetic thrusters aim for the highest possible efficiency and thrust. The choice of thruster will heavily depend on the specific mission parameters of the Exodus mission.
Ion Propulsion Integration into a Multi-Stage System
A multi-stage propulsion system for an Exodus mission would likely incorporate ion propulsion as a crucial component for the main interstellar cruise phase. The initial stages could utilize more powerful, but less efficient, propulsion systems like chemical rockets or perhaps nuclear thermal propulsion to achieve escape velocity and accelerate to a substantial fraction of the target velocity. Once the initial acceleration phase is complete, ion thrusters would take over, providing sustained low-thrust acceleration over many years or even decades, gradually building velocity to reach the destination. This staged approach would leverage the strengths of each propulsion technology, combining the high thrust of initial stages with the high efficiency of ion propulsion for the long-duration cruise phase. For example, a mission to Proxima Centauri might use chemical rockets for initial Earth escape and orbital maneuvers, followed by nuclear thermal propulsion for initial acceleration to a significant fraction of the target speed, then switching to ion propulsion for the decades-long cruise phase to minimize propellant mass. The precise design would depend on factors such as mission duration, target velocity, and available resources.
Solar Sails and Laser-Propelled Exodus
Solar sails and laser propulsion represent compelling alternatives to traditional rocket propulsion for interstellar voyages, offering the potential for faster and more efficient travel across vast distances. Both leverage the principles of momentum transfer, but differ significantly in their energy sources and operational mechanics. Understanding their strengths and weaknesses is crucial for evaluating their suitability for a large-scale exodus mission.
Solar sails utilize the pressure exerted by sunlight to propel a spacecraft. A large, highly reflective sail acts as a “light-sail,” capturing photons and converting their momentum into thrust. While seemingly minuscule, the cumulative effect of countless photons impacting the sail over extended periods can generate substantial acceleration, albeit at a gradual pace. Laser propulsion, conversely, employs powerful ground-based or space-based lasers to deliver concentrated beams of light onto a smaller, more robust sail, generating significantly higher thrust levels.
Solar Sail Mechanics and Applications for Exodus
Solar sails rely on the momentum transfer from photons striking a reflective surface. The force exerted is directly proportional to the sail’s reflectivity and inversely proportional to the square of the distance from the sun. This means thrust diminishes considerably as the spacecraft travels further from the sun. For an exodus mission, this implies a longer journey time, particularly for interstellar destinations. However, the advantage lies in the inherent simplicity and lack of onboard propellant. The sail itself is the only “fuel” required, making it attractive for long-duration missions where propellant mass is a significant constraint. Consider the hypothetical scenario of an exodus fleet utilizing solar sails: the larger the sail area per spacecraft, the slower but more consistent the acceleration would be, potentially allowing for more gradual and comfortable acceleration profiles for the passengers.
Laser Propulsion Mechanics and Applications for Exodus
Laser propulsion systems overcome the limitations of solar sails by using powerful lasers to provide a continuous and more intense light source. This allows for significantly higher acceleration compared to solar sails. The laser beam focuses its energy onto a relatively small, highly durable sail, resulting in a more focused and efficient transfer of momentum. For an exodus mission, this translates to shorter travel times and potentially more efficient use of resources. A potential challenge is the enormous energy requirements for the lasers themselves, necessitating substantial infrastructure on Earth or in space. Furthermore, maintaining a precise and continuous laser beam over interstellar distances presents a significant technological hurdle. Imagine a scenario where a network of space-based lasers relays the beam to the departing exodus fleet, ensuring a continuous energy supply for acceleration. This would, however, necessitate a significant investment in space-based infrastructure.
Comparison of Solar Sails and Laser Propulsion for Exodus Missions
Feature | Solar Sail | Laser Propulsion |
---|---|---|
Thrust | Low, gradually decreasing with distance | High, potentially constant with sufficient laser power |
Energy Source | Sunlight | Ground-based or space-based lasers |
Propellant | None (sail only) | None (sail only) |
Travel Time | Long | Shorter |
Technological Complexity | Relatively low | Relatively high |
Infrastructure Requirements | Minimal | Extensive (for laser systems) |
Scalability and Limitations of Solar Sails and Laser Propulsion for Interstellar Travel
Both solar sails and laser propulsion offer potential for scalability, though each faces unique limitations. Solar sails could be scaled up by increasing the sail area, but this introduces challenges in deployment and structural integrity. Laser propulsion scalability hinges on the development of more powerful and efficient lasers, as well as the ability to maintain a focused beam over vast distances. A key limitation for both systems is the energy requirement. For interstellar travel, enormous amounts of energy are needed, representing a significant hurdle to overcome. While theoretical calculations suggest the feasibility of interstellar travel using these methods, practical implementation faces substantial technological and engineering challenges. The development of advanced materials for the sails, capable of withstanding the immense stresses and radiation encountered during interstellar voyages, remains a critical area of research. Furthermore, the long-term reliability of such systems over decades-long journeys needs to be carefully considered.
Warp Drive and Alcubierre Metric: Exodus Propulsion Technologies
The concept of warp drive, allowing faster-than-light (FTL) travel, has captivated science fiction enthusiasts for decades. While currently relegated to the realm of theoretical physics, the Alcubierre metric provides a mathematical framework for exploring the possibility of such a drive. This framework, however, presents significant challenges that remain far beyond our current technological capabilities.
The Alcubierre drive proposes warping spacetime itself to achieve FTL travel. Instead of moving through space, the spacecraft would remain stationary within a “warp bubble,” a localized distortion of spacetime that contracts space in front of the bubble and expands it behind. This contraction and expansion effectively moves the bubble, and the spacecraft within it, at speeds exceeding the speed of light, without violating Einstein’s theory of special relativity within the bubble itself. This is because the spacecraft is not moving *through* space at superluminal speeds; rather, space itself is moving around the spacecraft.
Energy Requirements of the Alcubierre Drive
The energy demands of the Alcubierre drive are astronomical, posing a seemingly insurmountable hurdle. Calculations suggest that the energy required would be on the order of negative mass-energy, a hypothetical form of matter with negative mass. This is far beyond anything currently understood or observed in the universe. Even if negative mass-energy were to exist, the sheer magnitude of energy needed to generate and maintain the warp bubble would dwarf the total energy output of our sun, and likely many suns, over its entire lifetime. For example, if we were to consider the energy required to propel a small spacecraft, the equivalent energy might exceed the total energy output of a galaxy.
Potential Paradoxes and Causality Violations
The Alcubierre drive’s potential to violate causality, the principle that cause must precede effect, presents another significant concern. Faster-than-light travel opens the door to potential paradoxes, such as the possibility of time travel. A spacecraft traveling faster than light could potentially arrive at its destination before it departs, creating a situation where cause and effect are reversed. While the Alcubierre metric itself doesn’t inherently violate causality within the confines of the warp bubble, the implications of FTL travel raise serious questions about the fundamental structure of spacetime and the laws of physics. The potential for creating closed timelike curves (CTCs), which allow for time travel paradoxes, remains a significant theoretical challenge.
Scientific and Engineering Hurdles
Numerous scientific and engineering obstacles stand in the way of realizing a warp drive. Beyond the immense energy requirements, the very nature of negative mass-energy remains purely hypothetical. We lack any understanding of how to create or manipulate such a substance. Furthermore, the extreme spacetime curvature required to generate a warp bubble would necessitate precise control over exotic matter with properties currently unknown. The engineering challenges of constructing a spacecraft capable of withstanding the immense gravitational forces and stresses within a warp bubble are equally daunting, exceeding the capabilities of any foreseeable technology. Finally, the navigational complexities of maneuvering a warp bubble through interstellar space, avoiding collisions with celestial bodies and navigating unpredictable spacetime distortions, are also significant challenges.
Advanced Materials for Exodus Propulsion
The success of interstellar travel hinges critically on the development and utilization of advanced materials capable of withstanding the immense stresses and extreme environments encountered during such voyages. These materials must exhibit exceptional properties to ensure the structural integrity and operational efficiency of propulsion systems, shielding, and other crucial components. The following sections will delve into the necessary characteristics of these materials, explore promising candidates currently under investigation, and discuss the significant challenges associated with their large-scale production.
The extreme conditions of interstellar travel demand materials with unprecedented strength, durability, and resistance to various forms of degradation. These materials will face intense radiation, fluctuating temperatures spanning hundreds or thousands of degrees, and potentially high-energy particle impacts. Furthermore, the sheer duration of interstellar journeys necessitates materials with exceptional longevity and resistance to fatigue.
Material Properties for Interstellar Travel
Several key properties are paramount for materials destined for interstellar propulsion systems. These properties must be optimized simultaneously, which presents a significant materials science challenge.
- Extreme Temperature Tolerance: Materials must withstand both incredibly high temperatures (from fusion reactions or atmospheric re-entry) and extremely low temperatures (of deep space). This requires a wide operational temperature range and minimal thermal expansion.
- High Strength-to-Weight Ratio: Minimizing mass is crucial for interstellar travel, requiring materials with exceptional strength and stiffness relative to their weight. This is particularly important for propulsion system components.
- Radiation Resistance: Interstellar space is filled with high-energy radiation that can damage materials over time. Resistance to radiation-induced embrittlement and degradation is essential for long-duration missions.
- Impact Resistance: Collisions with micrometeoroids and space debris pose a significant risk. Materials must possess high impact resistance to prevent catastrophic damage.
- Self-Healing Capabilities: The ability of a material to repair minor damage autonomously would significantly enhance its longevity and reliability in the harsh environment of space.
Examples of Promising Advanced Materials
Research into advanced materials is constantly yielding new possibilities for interstellar propulsion. Several materials show great promise, though significant hurdles remain before they can be implemented at scale.
- Carbon Nanotubes and Graphene: These materials exhibit exceptional strength, flexibility, and thermal conductivity. Their lightweight nature makes them ideal candidates for many propulsion system components. However, challenges remain in producing large, defect-free sheets and integrating them into complex structures.
- Metallic Glasses: These amorphous alloys possess high strength, hardness, and corrosion resistance, surpassing many conventional metals. Their potential application lies in structural components requiring high durability and resistance to fatigue.
- High-Entropy Alloys: These alloys, composed of five or more principal elements in near-equiatomic proportions, demonstrate exceptional strength, ductility, and resistance to extreme temperatures. Their complex microstructure makes them particularly resistant to radiation damage.
- Superalloys with Advanced Coatings: Existing superalloys, already used in demanding applications like jet engines, can be enhanced with advanced coatings to improve their resistance to oxidation, corrosion, and erosion in the harsh space environment.
Challenges in Material Development and Manufacturing
Despite the exciting potential of these advanced materials, several challenges hinder their widespread adoption for interstellar travel.
Exodus propulsion technologies represent a significant challenge, demanding breakthroughs in various scientific fields. The successful development of these systems heavily relies on access to and effective utilization of crucial information or technology , ranging from advanced materials science to sophisticated computational modeling. Ultimately, the feasibility of interstellar travel hinges on our ability to master and integrate these diverse technological components.
- Cost of Production: Many advanced materials are currently expensive to produce, limiting their scalability for large-scale applications.
- Manufacturing Complexity: The intricate structures and precise properties of these materials often require complex and specialized manufacturing processes, which can be challenging to scale up.
- Long-Term Performance Prediction: Accurately predicting the long-term performance of these materials under the extreme conditions of interstellar space remains a significant challenge. Rigorous testing and modeling are crucial to ensure reliability.
- Material Degradation Mechanisms: A thorough understanding of the mechanisms by which these materials degrade under extreme conditions is necessary to develop strategies for mitigation and enhancement.
Energy Storage Solutions for Long-Duration Missions
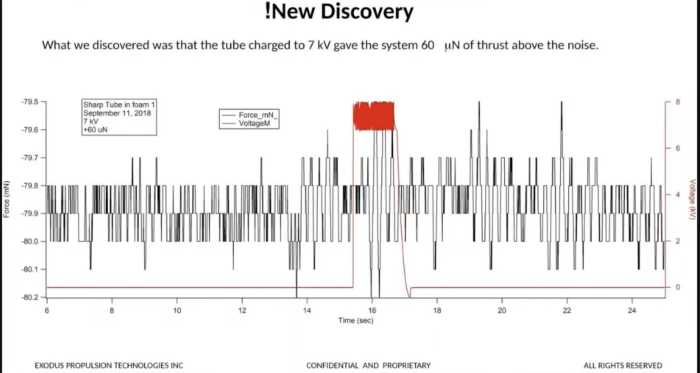
Powering a spacecraft for an interstellar exodus presents immense challenges. The journey’s duration necessitates energy storage solutions far exceeding current capabilities. This section compares various technologies, examines associated hurdles, and proposes a hypothetical energy management system for such a mission.
Long-duration space travel demands energy storage systems with high energy density, long lifespans, and the ability to withstand the rigors of interstellar space. Several technologies show promise, each with unique advantages and drawbacks. The selection of the optimal system depends on mission parameters, technological advancements, and resource availability.
Comparison of Energy Storage Technologies, Exodus propulsion technologies
The table below compares different energy storage technologies suitable for powering an exodus-style spacecraft. The metrics considered are energy density (energy stored per unit mass), power density (power output per unit mass), lifespan (expected operational lifetime), and technological maturity (current state of development and readiness).
Exodus propulsion technologies represent a significant challenge, demanding breakthroughs in various scientific fields. The successful development of these systems heavily relies on access to and effective utilization of crucial information or technology , ranging from advanced materials science to sophisticated computational modeling. Ultimately, the feasibility of interstellar travel hinges on our ability to master and integrate these diverse technological components.
Technology | Energy Density (kWh/kg) | Power Density (kW/kg) | Lifespan (Years) | Technological Maturity |
---|---|---|---|---|
Nuclear Fission | Very High (depending on reactor design) | High | Decades | High |
Nuclear Fusion | Extremely High (potential) | Very High (potential) | Decades (potential) | Medium-Low (under development) |
Advanced Batteries (e.g., Lithium-sulfur, solid-state) | Medium | Medium-High | 5-10 | Medium |
Fuel Cells (e.g., hydrogen fuel cells) | Medium (depending on fuel storage) | Medium | Variable, depends on fuel supply | High |
Challenges in Energy Storage and Management During Interstellar Journeys
Several significant challenges must be addressed for effective energy storage and management during long interstellar journeys. These include:
- Energy Density Requirements: Interstellar travel demands extremely high energy density to minimize the spacecraft’s mass, which directly impacts propulsion efficiency and overall mission feasibility. Current technologies fall short of the necessary levels.
- System Lifespan and Reliability: The mission’s duration requires energy storage systems with exceptionally long lifespans and high reliability. Failures in space are costly and often irreparable.
- Radiation Hardening: The harsh radiation environment of interstellar space can degrade energy storage systems. Components must be designed to withstand significant radiation exposure.
- Thermal Management: Efficient thermal management is crucial to maintain optimal operating temperatures for the energy storage and power generation systems, especially during prolonged periods of operation.
- Energy Conversion Efficiency: The efficiency of converting stored energy into usable power significantly impacts the overall energy budget. Minimizing energy losses is essential.
Hypothetical Energy Management System for an Exodus Mission
A viable energy management system for an exodus mission would likely incorporate a hybrid approach, combining multiple energy storage and generation technologies. For example:
A system could use a nuclear fission reactor as the primary power source, providing a continuous and reliable supply of energy. Advanced batteries could act as a buffer, storing energy during periods of high power demand or when the reactor is undergoing maintenance. Fuel cells could provide supplemental power, utilizing fuel produced or collected during the journey. Sophisticated power management algorithms would optimize energy distribution to various spacecraft systems, ensuring efficient usage and minimizing waste.
This hybrid system would leverage the strengths of each technology, mitigating their individual weaknesses. The continuous power supply from the fission reactor would be complemented by the high power density of the batteries for short bursts of high energy consumption, while fuel cells would offer a supplementary and flexible energy source. Such a system, along with advanced thermal management and radiation shielding, could potentially support a long-duration interstellar mission.
Life Support Systems and Long-Term Space Habitation
The success of any long-duration space mission, particularly an exodus-scale undertaking, hinges critically on the ability to create and maintain a self-sustaining environment capable of supporting human life for extended periods. This requires sophisticated life support systems that effectively recycle resources and mitigate the harsh realities of space. The challenges are immense, demanding innovative technological solutions and a deep understanding of human physiology and psychology.
A closed-loop life support system is essential for long-duration space travel, minimizing reliance on resupply from Earth. This system must manage several critical functions simultaneously to ensure crew survival and well-being.
Critical Components of a Closed-Loop Life Support System
Several interconnected systems must work in harmony to create a habitable environment. Failure in any one area could have catastrophic consequences for the mission.
- Atmosphere Control: This involves regulating the levels of oxygen, carbon dioxide, and other gases within the habitat. This typically includes oxygen generation (e.g., through electrolysis of water), carbon dioxide removal (e.g., using chemical scrubbers or biological systems), and control of humidity and temperature.
- Water Recycling: Recycling wastewater from showers, sinks, and other sources is crucial to conserve resources. This involves multiple stages of filtration and purification to ensure potable water is available.
- Waste Management: Effective waste management is critical, encompassing the processing of human waste, food waste, and other refuse. This often involves composting and other biological processes to minimize waste volume and potentially generate useful byproducts.
- Food Production: Growing food in space, through hydroponics or aeroponics, is vital for long-duration missions to reduce reliance on pre-packaged supplies. This requires careful control of light, nutrients, and other environmental factors.
- Radiation Shielding: Protecting the crew from harmful radiation from solar flares and cosmic rays is paramount. This might involve using specialized materials, habitat design, or a combination of both.
Psychological and Physiological Challenges of Long-Term Space Travel
The physiological and psychological effects of prolonged space travel pose significant risks to astronaut health and mission success. These challenges necessitate proactive measures to mitigate their impact.
Physiological challenges include bone density loss due to microgravity, muscle atrophy, cardiovascular deconditioning, and the increased risk of radiation exposure. Psychological challenges can manifest as stress, anxiety, depression, sleep disturbances, and interpersonal conflicts within the confined crew environment. The prolonged isolation and confinement inherent in long-duration space missions can significantly exacerbate these issues.
Maintaining Crew Health and Well-being During an Exodus Mission
A comprehensive plan is necessary to address both the physiological and psychological challenges of a long-duration space mission. This plan must be multifaceted and proactive.
- Countermeasures for Physiological Effects: Regular exercise regimens, including resistance training and cardiovascular activity, are essential to combat muscle atrophy and bone density loss. Nutritional strategies should focus on maintaining bone health and preventing other deficiencies. Pharmacological interventions may also be necessary to manage specific health risks.
- Psychological Support: A robust psychological support program is critical, involving pre-flight psychological screening and training, ongoing counseling and support from onboard psychologists or tele-psychologists, and strategies to promote crew cohesion and morale. This may include activities designed to reduce stress, maintain social connections, and foster a sense of community among crew members.
- Environmental Design: The design of the space habitat plays a vital role in maintaining crew well-being. The environment should be designed to maximize natural light exposure, minimize noise pollution, and provide opportunities for privacy and relaxation. The inclusion of recreational areas and opportunities for creative expression can help maintain morale and mental well-being.
- Regular Health Monitoring: Continuous health monitoring is crucial to detect and address health issues promptly. This involves regular medical check-ups, physiological data collection, and the use of telemedicine technologies to consult with specialists on Earth.
- Emergency Preparedness: A detailed emergency plan should address potential medical emergencies, equipment failures, and other unforeseen events. This plan should include protocols for medical treatment, emergency repairs, and communication with ground control.
Closing Notes
The pursuit of exodus propulsion technologies embodies humanity’s inherent drive to explore and expand beyond the confines of our solar system. While significant hurdles remain, the innovative concepts and ongoing research discussed herein demonstrate the remarkable progress being made. From the refinement of existing propulsion methods to the exploration of groundbreaking theoretical possibilities, the path toward interstellar travel is gradually becoming clearer, promising an exciting future where the stars are within our reach. Continued investment in research and development is crucial to overcoming the remaining challenges and realizing the dream of interstellar exploration.