Water energy technology offers a compelling pathway towards sustainable energy production. Harnessing the immense power of water, whether through traditional hydropower or innovative ocean energy conversion systems, presents a significant opportunity to diversify energy portfolios and mitigate climate change. This exploration delves into the diverse methods of generating power from water, examining their technological intricacies, environmental impacts, and economic viability. We will uncover the potential of hydropower, wave energy, tidal energy, and ocean thermal energy conversion, as well as the crucial role of water-based energy storage solutions.
From the engineering marvels of large-scale hydropower dams to the subtle mechanics of wave energy converters, this discussion will illuminate the breadth and depth of this vital field. We will also consider the challenges inherent in these technologies, such as environmental impacts and economic considerations, while highlighting recent advancements and future trends that promise to revolutionize the energy landscape.
Hydropower Generation Technologies
Hydropower, harnessing the energy of flowing water, represents a significant source of renewable energy. Different technologies exist, each with its own advantages and disadvantages concerning efficiency, environmental impact, and cost. Understanding these variations is crucial for responsible hydropower development.
Types of Hydropower Plants
Hydropower plants are categorized based on their design and operational characteristics. Three primary types are run-of-river, impoundment, and pumped storage hydropower plants. Each type offers a unique balance between energy production, environmental considerations, and economic viability.
Type | Efficiency | Environmental Impact | Cost |
---|---|---|---|
Run-of-River | Generally lower; dependent on natural water flow | Lower environmental impact compared to impoundment; minimal disruption to river flow; potential for fish migration issues. | Lower initial investment cost; higher operational costs in periods of low water flow. |
Impoundment | Higher; consistent energy generation due to reservoir storage | Higher environmental impact; significant alteration of river ecosystems; potential for greenhouse gas emissions from decaying organic matter in reservoirs; displacement of communities and habitats. | Higher initial investment cost; lower operational costs due to consistent energy generation. |
Pumped Storage | Lower net efficiency; energy is consumed during pumping | Moderate environmental impact; reservoir construction impacts; potential for water quality changes. | High initial investment cost; valuable for grid stability and peak demand management. |
Engineering Design Considerations for Hydropower Dams
The design of a modern hydropower dam requires meticulous planning and engineering expertise to ensure structural integrity and long-term performance. Material selection is crucial, balancing strength, durability, and cost-effectiveness. Concrete remains the dominant material, but advancements in composite materials are being explored for specific applications. Geotechnical investigations are essential to assess the stability of the dam foundation and surrounding geology, considering factors such as seismic activity and potential ground movements. Sophisticated computer modelling and simulations are used to analyze stress distributions within the dam structure under various loading conditions. Regular inspections and maintenance are crucial for the long-term safety and efficiency of the dam.
Feasibility Assessment of a New Hydropower Project
A comprehensive feasibility study is vital before initiating a new hydropower project. This involves a systematic evaluation of technical, economic, and environmental factors.
- Site Selection: This involves identifying potential locations with sufficient water flow, suitable topography, and minimal environmental impact. Factors such as proximity to transmission lines and population centers are also considered.
- Hydrological Studies: Detailed analysis of water flow patterns, seasonal variations, and potential for droughts or floods is conducted to estimate energy generation capacity.
- Environmental Impact Assessment: This crucial step assesses the potential effects of the project on aquatic ecosystems, terrestrial habitats, and local communities. Mitigation measures are identified and incorporated into the project design.
- Engineering Design and Cost Estimation: Detailed engineering designs are developed, including dam structure, power plant, and transmission lines. A comprehensive cost estimate is prepared, encompassing construction, operation, and maintenance.
- Economic Analysis: The financial viability of the project is evaluated, considering factors such as energy prices, project lifespan, and potential revenue streams.
- Regulatory Approvals: Necessary permits and approvals from relevant authorities are obtained, ensuring compliance with environmental regulations and safety standards.
Ocean Energy Conversion Technologies
Ocean energy, encompassing wave, tidal, and ocean thermal energy conversion (OTEC) technologies, presents a significant, albeit challenging, opportunity for sustainable energy generation. These methods leverage the immense power contained within the world’s oceans, offering a diverse portfolio of renewable energy sources to complement existing strategies. The following sections will explore the individual technologies, their comparative advantages and disadvantages, and the obstacles to widespread adoption.
Comparison of Wave, Tidal, and OTEC Systems
The three primary methods for harnessing ocean energy each present unique characteristics and challenges. Understanding these differences is crucial for optimizing their deployment and maximizing their potential contribution to the global energy mix.
- Wave Energy Converters (WECs): WECs capture the kinetic energy of surface waves through various mechanisms, including oscillating water columns, point absorbers, and overtopping devices. Their energy output is highly variable, dependent on wave height and frequency, but they can be deployed in a wide range of coastal locations. WECs are generally less predictable than tidal energy systems but require less initial investment in infrastructure.
- Tidal Energy Converters (TECs): TECs utilize the predictable rise and fall of tides to generate electricity. These systems, typically employing barrage systems or tidal stream turbines, offer a higher degree of predictability than WECs. However, suitable locations for TECs are geographically limited to areas with significant tidal ranges, and the associated environmental impacts require careful consideration. Furthermore, the high initial capital costs can be a barrier to widespread adoption.
- Ocean Thermal Energy Conversion (OTEC): OTEC systems exploit the temperature difference between warm surface water and cold deep ocean water to drive a power cycle. This technology is unique in its ability to generate power continuously, independent of wave or tidal patterns. However, OTEC requires substantial infrastructure and significant capital investment, and is only feasible in tropical regions with sufficient temperature gradients.
Challenges in Harnessing Ocean Energy
The marine environment presents several significant engineering and operational challenges for ocean energy technologies. These challenges necessitate robust and innovative designs to ensure long-term reliability and cost-effectiveness.
- Corrosion: The constant exposure to saltwater leads to rapid corrosion of many materials commonly used in energy generation systems. This necessitates the use of specialized corrosion-resistant alloys and coatings, adding to the overall cost and complexity of the technology.
- Marine Growth: Biofouling, the accumulation of marine organisms on submerged components, can significantly reduce the efficiency of ocean energy converters. Regular cleaning and maintenance are required, which are both time-consuming and expensive. Anti-fouling technologies are continuously being developed to mitigate this problem.
- Unpredictable Wave Patterns: The power output of wave energy converters is directly affected by the variability of wave patterns. This requires sophisticated control systems and energy storage solutions to ensure a consistent power supply. Advanced forecasting techniques are also being employed to improve predictability.
Conceptual Model for a Hybrid Ocean Energy System
A hybrid system combining wave and tidal energy technologies could offer improved efficiency and reliability. For instance, a coastal installation could utilize wave energy converters positioned near the shoreline to capture wave energy, while tidal stream turbines could be deployed in a nearby channel to harness tidal currents. The combined energy output from both systems would be fed into a common grid, enhancing the overall energy yield and reducing reliance on a single energy source. This system would benefit from the relatively lower initial investment of WECs and the higher predictability of TECs, mitigating some of the individual limitations of each technology. Such a system could be optimized using advanced energy management strategies and sophisticated control algorithms, maximizing energy capture and grid stability. The system could incorporate advanced energy storage, such as pumped hydro storage or battery banks, to address the intermittency of wave energy. Furthermore, real-time monitoring and predictive modelling could optimize energy extraction based on environmental conditions. The specific design would depend on the site’s characteristics, including wave climate, tidal range, and available space.
Water Energy Storage Technologies
Water energy storage plays a crucial role in the transition to a sustainable energy future, particularly in addressing the intermittency of renewable sources like solar and wind power. Effective energy storage allows for a more reliable and stable electricity grid, enhancing the integration of these renewable energy sources and improving overall grid efficiency. This section will explore various water-based energy storage technologies, focusing on their capabilities and limitations.
Pumped Hydro Storage and Grid Stability
Pumped hydro storage (PHS) is currently the dominant large-scale energy storage technology. It leverages the potential energy of water stored at a higher elevation to generate electricity during peak demand periods. During periods of low energy demand or surplus renewable energy generation, excess electricity is used to pump water back up to the higher reservoir. This cyclical process allows for efficient storage and retrieval of energy, significantly contributing to grid stability. PHS systems provide a rapid response to fluctuations in electricity demand, acting as a crucial buffer against intermittent renewable energy sources and ensuring grid reliability. The large-scale energy storage capacity of PHS systems enables the integration of substantial amounts of renewable energy into the electricity grid, facilitating a smoother transition towards a decarbonized energy sector.
Comparison of Pumped Hydro Storage with Other Energy Storage Technologies
The following table compares the advantages and disadvantages of pumped hydro storage with other prominent energy storage technologies:
Feature | Pumped Hydro Storage | Batteries | Compressed Air Energy Storage (CAES) |
---|---|---|---|
Energy Capacity | Very High (GW-scale) | Medium to High (MW-scale) | Medium (MW-scale) |
Duration | Long (hours to days) | Short to Medium (minutes to hours) | Medium (hours) |
Efficiency | 70-80% | 80-90% | 50-70% |
Cost | High initial investment, low operating cost | High initial and operating costs (decreasing) | Medium to high initial investment, medium operating cost |
Environmental Impact | Moderate (land use, water consumption) | Moderate (material sourcing, manufacturing, disposal) | Low to moderate (land use, potential for groundwater contamination) |
Scalability | High | High (but geographically limited by material supply chains) | Medium |
Types of Pumped Hydro Storage Systems and Suitability
Several types of pumped hydro storage systems exist, each with varying suitability depending on geographical location and energy demands. Open-loop systems utilize two separate reservoirs, while closed-loop systems use a single reservoir and a lower basin. Closed-loop systems are often more suitable for locations with limited water resources or challenging topography. Another variation is the reversible pump-turbine system which combines pumping and generation functions within a single unit, increasing efficiency and reducing costs. The choice of system depends heavily on site-specific factors, including elevation difference, available water resources, geological conditions, and environmental considerations. For instance, open-loop systems are best suited to areas with significant elevation differences and abundant water resources, while closed-loop systems are better suited to areas with limited water availability or where environmental impacts need to be minimized.
Innovative Water-Based Energy Storage Technologies
Beyond traditional pumped hydro, several innovative water-based energy storage technologies are emerging. One example is compressed air energy storage (CAES) with water. This technology combines the principles of compressed air energy storage with the thermal properties of water. During off-peak hours, excess electricity compresses air, which is then stored in underground caverns. The compressed air can be used to drive turbines during peak demand periods, generating electricity. The integration of water enhances the efficiency of the system by storing and releasing heat, improving the overall energy conversion process. This technology offers a potential alternative to traditional PHS, especially in regions where suitable geographical conditions for PHS are limited. The use of water in CAES can mitigate some of the efficiency losses associated with conventional CAES systems. Another example is the use of hydro-pneumatic accumulators, which utilize the pressure of compressed air and water to store energy and provide fast response times, ideal for applications requiring rapid energy delivery.
Water Desalination and Energy Production
Desalination, the process of removing salts and minerals from seawater or brackish water to produce freshwater, is a crucial technology for addressing water scarcity in many parts of the world. However, the energy intensity of desalination is a significant concern, impacting both its economic viability and environmental footprint. This section explores different desalination methods, their energy consumption, and strategies for minimizing their environmental impact.
Several methods exist for desalination, each with varying energy requirements and environmental consequences. The most common are thermal methods, such as multi-stage flash (MSF) and multi-effect distillation (MED), and membrane-based methods, primarily reverse osmosis (RO). Thermal methods involve boiling seawater to produce steam, which is then condensed to yield freshwater. Membrane-based methods, on the other hand, use pressure to force seawater through a semi-permeable membrane, leaving the salts behind.
Desalination Methods: Energy Efficiency and Environmental Impact
The energy efficiency and environmental impact of different desalination methods vary considerably. Reverse osmosis, while generally more energy-efficient than thermal methods, still consumes significant energy, primarily for high-pressure pumping. Thermal methods, although less efficient, can utilize waste heat from other industrial processes, potentially reducing their overall energy consumption. The environmental impact includes energy consumption, brine discharge (highly concentrated salt water), and the use of chemicals for pretreatment and membrane cleaning.
Method | Energy Consumption (kWh/m³) | Environmental Impact |
---|---|---|
Reverse Osmosis (RO) | 3-5 | Lower energy consumption than thermal methods, but brine discharge and chemical use remain concerns. |
Multi-Stage Flash (MSF) | 10-15 | High energy consumption, significant thermal pollution from cooling water discharge. |
Multi-Effect Distillation (MED) | 6-12 | Lower energy consumption than MSF, but still relatively high compared to RO; brine discharge remains a concern. |
Note: Energy consumption values are approximate and can vary depending on factors such as water salinity, plant size, and operating conditions. Environmental impacts are summarized and require further detailed analysis for a complete assessment.
Integrating Desalination with Renewable Energy Sources
To reduce the carbon footprint of desalination, integrating it with renewable energy sources, such as solar, wind, and geothermal energy, is crucial. This can significantly reduce reliance on fossil fuels and lower greenhouse gas emissions. For example, a desalination plant powered by solar photovoltaic (PV) panels can reduce its reliance on the grid and minimize its carbon footprint. Similarly, wind energy can provide a reliable power source in areas with consistent wind resources. Hybrid systems combining different renewable energy sources can further enhance reliability and sustainability. The Israeli Sorek desalination plant, for instance, is a large-scale example of integrating renewable energy sources, although it still uses some fossil fuels.
Utilizing Waste Heat from Desalination Plants
Desalination plants generate significant amounts of waste heat during operation, particularly thermal methods. This waste heat can be harnessed for various purposes, such as district heating, reducing overall energy consumption and improving energy efficiency. The heat can be used to warm buildings, providing a sustainable and cost-effective heating solution. This approach contributes to energy recovery and minimizes environmental impact by reducing the need for conventional heating systems. Several pilot projects worldwide demonstrate the feasibility of utilizing waste heat from desalination for district heating, showcasing its potential for sustainable development.
Environmental Impacts of Water Energy Technologies
Harnessing the power of water, whether from rivers, oceans, or reservoirs, offers a significant opportunity for renewable energy generation. However, these technologies are not without their environmental consequences. Understanding and mitigating these impacts is crucial for ensuring the sustainable development and deployment of water-based energy solutions. The following sections detail the ecological considerations associated with various water energy technologies.
Ecological Impacts of Hydropower Development
Hydropower, while a clean energy source, can significantly alter aquatic ecosystems. The construction of dams fragments river habitats, isolating fish populations and disrupting natural migration patterns. For example, the construction of the Three Gorges Dam in China resulted in the displacement of numerous species and altered the downstream flow regime, impacting biodiversity and sediment transport. Changes in water flow regimes, caused by dam operation, can also affect water temperature, dissolved oxygen levels, and the overall health of the river ecosystem. These changes can lead to habitat loss, reduced species diversity, and altered community structures. The alteration of sediment transport can lead to erosion in some areas and sedimentation in others, further impacting the aquatic environment.
Environmental Impacts of Ocean Energy Technologies
Ocean energy technologies, including wave and tidal energy converters, also present potential environmental challenges. Noise pollution from the operation of these devices can affect marine mammals, fish, and other marine life, potentially disrupting communication, navigation, and foraging behaviors. The physical presence of these structures can also alter marine habitats, affecting benthic communities and potentially leading to habitat loss. Furthermore, the potential for collisions between marine animals and these structures is a concern, particularly for larger species such as whales and sea turtles. Studies on the effects of wave energy converters on marine life are still ongoing, and long-term monitoring is essential to fully understand their impact.
Mitigation Strategies for Minimizing Negative Environmental Effects
Minimizing the negative environmental impacts of water energy technologies requires a proactive and multi-faceted approach. Effective mitigation strategies are essential for ensuring the sustainable development of these energy sources.
- Careful Site Selection and Environmental Impact Assessments: Thorough assessments are crucial to identify and minimize potential impacts on sensitive ecosystems. This includes considering the presence of endangered species, important habitats, and potential cumulative effects.
- Fish Passage Solutions: Implementing fish ladders, fish bypasses, or other fish passage structures can help maintain fish migration routes and reduce habitat fragmentation. The design and effectiveness of these structures must be carefully considered based on the specific species and river conditions.
- Modified Dam Operations: Adjusting dam operations to mimic natural flow regimes can help mitigate the negative impacts on downstream ecosystems. This may involve controlled releases of water to simulate natural fluctuations and maintain essential ecological processes.
- Environmental Monitoring: Ongoing monitoring of water quality, fish populations, and other ecological indicators is necessary to track the effectiveness of mitigation measures and identify any unforeseen impacts. This allows for adaptive management strategies to be implemented as needed.
- Marine Habitat Protection Measures: For ocean energy technologies, strategies to minimize noise pollution, such as using quieter technologies or implementing noise reduction measures, are essential. Careful siting to avoid sensitive habitats and the implementation of design features to minimize impacts on marine life are also important considerations.
Economic Aspects of Water Energy Technologies
The economic viability of water energy technologies is a crucial factor influencing their widespread adoption. Factors such as initial investment costs, operational expenses, and long-term profitability significantly impact the attractiveness of these projects for investors and governments. A comprehensive economic analysis needs to consider the entire lifecycle of a project, from planning and construction to operation and decommissioning.
Levelized Cost of Energy Comparison
The levelized cost of energy (LCOE) provides a standardized metric for comparing the cost-effectiveness of different electricity generation technologies. It represents the average cost per unit of electricity generated over the entire lifespan of a project, considering all costs and discounting them to present value. Lower LCOE values indicate greater economic competitiveness. The following table presents a comparative analysis of LCOE for various water energy technologies. Note that these values are estimates and can vary significantly based on specific project characteristics and geographical location.
Technology | LCOE (USD/MWh) – Low Estimate | LCOE (USD/MWh) – Mid Estimate | LCOE (USD/MWh) – High Estimate |
---|---|---|---|
Hydropower (Large Dam) | 40 | 60 | 80 |
Hydropower (Run-of-River) | 50 | 70 | 90 |
Tidal Energy | 100 | 150 | 200 |
Wave Energy | 150 | 200 | 250 |
Ocean Thermal Energy Conversion (OTEC) | 200 | 300 | 400 |
Economic Feasibility in Different Regions
The economic feasibility of water energy projects varies considerably across different regions due to diverse factors. Resource availability plays a dominant role; areas with abundant hydropower potential, strong tidal currents, or significant wave energy resources will naturally find these technologies more economically attractive. Infrastructure costs, including transmission lines and access roads, can significantly impact project economics, especially in remote locations. Government policies, such as subsidies, tax incentives, and regulatory frameworks, also influence the financial viability of water energy projects. For example, regions with supportive policies and streamlined permitting processes tend to attract more investment.
Examples of Successful Water Energy Projects
The Itaipu Dam on the border of Brazil and Paraguay exemplifies a highly successful large-scale hydropower project. Its immense power generation capacity, coupled with effective management and long-term planning, has delivered significant economic benefits to both countries. The success of Itaipu can be attributed to factors such as favorable geological conditions, robust infrastructure, and strong governmental support. Conversely, the failure of some smaller-scale projects can often be traced to inadequate planning, cost overruns, and insufficient consideration of environmental factors. A thorough feasibility study incorporating all relevant economic, environmental, and social factors is crucial for the long-term success of any water energy project.
Technological Advancements in Water Energy
The field of water energy is experiencing a period of rapid technological advancement, driven by the increasing demand for sustainable and renewable energy sources. Innovations in materials science, computational fluid dynamics, and control systems are leading to significant improvements in the efficiency, durability, and overall performance of water energy technologies. This progress is crucial for maximizing energy extraction, minimizing environmental impact, and ensuring the long-term viability of these crucial energy resources.
Recent advancements are not only increasing the efficiency of existing technologies but also enabling the development of entirely new approaches to harnessing water’s energy potential. These developments are paving the way for more widespread adoption of water energy and a greater contribution to global energy security.
Advancements in Hydropower Turbine Technology
The quest for higher efficiency and durability in hydropower turbines has led to the development of several innovative designs and materials. For instance, the use of advanced computational fluid dynamics (CFD) modeling allows engineers to optimize turbine blade geometries for improved energy capture. This results in turbines that extract more energy from the water flow, increasing overall power output and reducing the environmental footprint by requiring fewer turbines to generate the same amount of power. Furthermore, the incorporation of new materials, such as high-strength composites and corrosion-resistant alloys, enhances the lifespan and reliability of turbines, minimizing maintenance needs and downtime. Specific examples include the development of more efficient Kaplan turbines with optimized blade designs and the use of advanced materials in Francis turbines to withstand high pressures and erosive forces. These advancements significantly improve the economic viability of hydropower projects.
Innovative Materials and Designs in Ocean Energy Converters
Ocean energy conversion technologies, such as wave energy converters and tidal energy generators, face unique challenges due to the harsh marine environment. Therefore, the development of robust and durable materials is paramount. Recent advancements include the use of high-strength polymers, reinforced composites, and corrosion-resistant coatings to protect these devices from saltwater corrosion, biofouling, and wave impacts. Innovative designs, such as flexible wave energy converters that adapt to changing wave conditions and modular tidal turbine arrays that optimize energy capture across a range of tidal flows, are also being developed. For example, some wave energy converters now utilize flexible structures that can adapt to various wave heights and periods, enhancing energy capture efficiency and reducing structural fatigue. Similarly, advances in materials science have allowed for the creation of more resilient components capable of withstanding the constant stress of ocean currents.
Emerging Water Energy Technologies, Water energy technology
Beyond improvements to existing technologies, several emerging water energy technologies hold significant promise. One such technology is osmotic power, which harnesses the energy generated by the difference in salinity between freshwater and saltwater. This technology, still in its early stages of development, could potentially provide a significant source of clean energy, particularly in coastal regions. Another promising area is the development of improved systems for harnessing the energy from ocean currents, which can be quite powerful and consistent. This approach could potentially provide a reliable baseload energy source, supplementing intermittent renewable energy sources like solar and wind. Furthermore, research is ongoing in exploring the potential of utilizing wave energy to drive desalination processes, creating a synergistic system that provides both clean water and energy. The development of more efficient and cost-effective technologies in these areas is crucial for their future widespread adoption. Early pilot projects for osmotic power and enhanced ocean current energy systems are showing promising results, suggesting that these technologies could become commercially viable in the near future.
Policy and Regulatory Frameworks for Water Energy
Government policies and regulations play a crucial role in shaping the development and deployment of water energy technologies. Effective policies can stimulate investment, accelerate innovation, and ensure the sustainable and responsible use of water resources for energy production. Conversely, inadequate or inconsistent regulations can hinder progress and lead to environmental damage or social inequities. The global landscape of water energy policy is diverse, reflecting varying national priorities, resource availability, and environmental concerns.
The success of water energy projects hinges significantly on supportive policy frameworks. These frameworks must address issues such as permitting processes, environmental impact assessments, grid connection, and financial incentives. Furthermore, they need to consider the social implications of projects, ensuring equitable distribution of benefits and mitigation of potential negative impacts on communities.
Regulatory Frameworks for Water Energy Across Countries
The regulatory landscape for water energy varies considerably across nations. Differences in legal systems, political priorities, and levels of technological development all contribute to this diversity. A consistent and supportive regulatory environment is crucial for attracting investment and fostering innovation in the water energy sector.
- United States: The US employs a complex, multi-agency regulatory approach involving federal, state, and local authorities. The Federal Energy Regulatory Commission (FERC) primarily regulates hydropower projects, while other agencies like the Environmental Protection Agency (EPA) oversee environmental compliance. State-level regulations often govern smaller-scale projects and water rights.
- European Union: The EU promotes a more harmonized approach through directives and regulations aimed at achieving energy security and environmental protection. The Water Framework Directive and the Renewable Energy Directive influence the development and licensing of water energy projects. Individual member states retain significant autonomy in implementing these directives.
- China: China has actively promoted hydropower development through national policies and investment programs. The government plays a dominant role in planning, financing, and regulating large-scale hydropower projects, prioritizing national energy security goals.
- Brazil: Brazil possesses significant hydropower capacity and has a relatively mature regulatory framework. However, ongoing challenges include balancing energy production with environmental protection and the needs of indigenous communities.
Hypothetical Policy Proposal: Incentivizing Water Energy Investment
This proposal aims to stimulate investment in water energy projects through a combination of financial incentives and streamlined regulatory processes.
The core components include:
- Tax Credits and Subsidies: Offering significant tax credits for the development and deployment of new water energy technologies, particularly those focusing on wave, tidal, and osmotic power. Subsidies could be provided for research and development, as well as for the construction and operation of demonstration projects.
- Streamlined Permitting Processes: Establishing a centralized permitting agency to expedite the approval of water energy projects, minimizing bureaucratic hurdles and reducing processing times. This agency would also provide clear guidance and standardized procedures to enhance transparency and predictability.
- Guaranteed Power Purchase Agreements (PPAs): Governments could guarantee PPAs for water energy projects, ensuring a stable revenue stream for investors and reducing investment risk. This would attract private investment, crucial for large-scale deployment.
- Investment in Research and Development (R&D): Dedicated funding for R&D to advance the technological capabilities of water energy, particularly in areas such as improving efficiency, reducing environmental impacts, and developing cost-effective technologies.
- Public Awareness Campaigns: Promoting public awareness of the benefits of water energy through educational campaigns, highlighting its potential for clean energy production and its contribution to climate change mitigation.
Successful implementation of this proposal requires strong inter-agency coordination, effective monitoring and evaluation mechanisms, and ongoing stakeholder engagement to ensure its effectiveness and address any unintended consequences.
Future Trends in Water Energy Technology
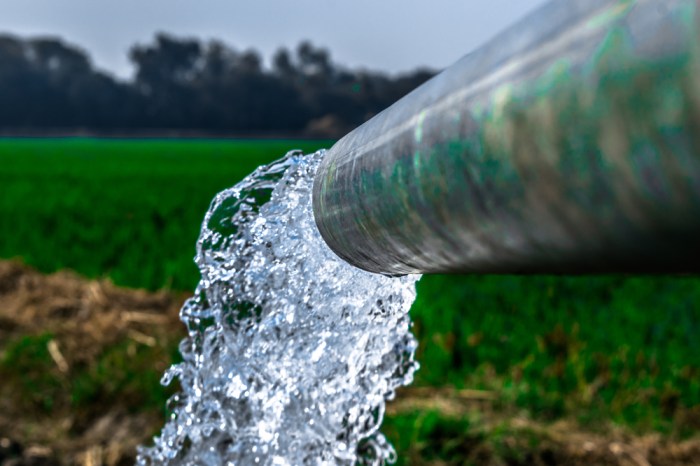
The future of water energy hinges on its ability to integrate seamlessly with existing and emerging energy infrastructure, enhance efficiency through advanced technologies, and build resilience against the impacts of climate change. This requires a multifaceted approach encompassing smart grid integration, AI-driven optimization, and a redesigned approach to water resource management.
The potential for significant advancements in water energy technology is substantial, driven by both technological innovation and the increasing urgency to address climate change and energy security concerns. These advancements promise more efficient, sustainable, and resilient water energy systems that can contribute significantly to a global transition to cleaner energy sources.
Integration with Smart Grids and Renewable Energy Sources
Integrating water energy technologies, particularly hydropower and wave energy converters, with smart grids offers substantial benefits. Smart grids provide the infrastructure for real-time monitoring and control of energy generation and distribution, allowing for optimal dispatch of water-based energy based on demand and resource availability. This integration can effectively balance intermittent renewable energy sources like solar and wind power, smoothing out fluctuations in supply and improving grid stability. For example, pumped hydro storage, a form of water energy storage, can store excess energy from solar and wind farms during periods of high generation and release it during peak demand periods. This enhances the reliability and efficiency of the entire renewable energy system. Furthermore, advanced grid management systems can predict and manage water resource availability for hydropower generation, maximizing energy output while minimizing environmental impacts.
The Role of Artificial Intelligence and Machine Learning in Optimizing Water Energy Systems
Artificial intelligence (AI) and machine learning (ML) are poised to revolutionize the operation and management of water energy systems. AI-powered predictive models can forecast water availability, energy demand, and system performance, enabling proactive adjustments to optimize energy generation and minimize waste. For instance, ML algorithms can analyze historical data and real-time sensor readings to predict optimal turbine operation parameters for hydropower plants, maximizing energy output while minimizing wear and tear on equipment. Similarly, AI can be used to monitor and predict potential failures in water energy infrastructure, allowing for timely maintenance and reducing downtime. This proactive approach to system management not only enhances efficiency but also improves the overall lifespan and reliability of water energy infrastructure. The use of AI in desalination plants can also optimize the energy consumption and brine management, leading to a more sustainable and efficient process.
A Conceptual Framework for a Sustainable, Efficient, and Climate-Resilient Water Energy System
A future water energy system must be designed with sustainability, efficiency, and climate resilience at its core. This requires a holistic approach that considers the entire water cycle, from resource management to energy generation and distribution. The framework should prioritize decentralized generation wherever feasible, reducing transmission losses and improving local energy security. It should also integrate diverse water energy technologies, such as hydropower, wave energy, tidal energy, and osmotic power, to create a diversified and resilient energy portfolio. This diversification mitigates the risk associated with reliance on a single technology and enhances overall system stability. Furthermore, the system should incorporate advanced water management techniques to minimize water consumption and environmental impacts, ensuring sustainable water resource utilization. This might include rainwater harvesting, water reuse strategies, and efficient irrigation techniques. Finally, the framework must account for the impacts of climate change, such as altered precipitation patterns and sea-level rise, ensuring the long-term viability and resilience of the water energy system. This may involve the development of climate-resilient infrastructure and adaptive management strategies.
Final Conclusion: Water Energy Technology
In conclusion, water energy technology stands as a crucial component of a sustainable energy future. While challenges remain in optimizing efficiency, minimizing environmental impact, and ensuring economic feasibility, ongoing innovation and strategic policy decisions are paving the way for widespread adoption. The diverse range of technologies discussed, from established hydropower to emerging ocean energy solutions, offers a multifaceted approach to harnessing water’s potential. By embracing a holistic perspective that considers technological advancements, environmental stewardship, and economic viability, we can unlock the full potential of water energy and create a cleaner, more sustainable world.